Stolper Research Interests
The influence of phase equilibria in peridotites on the oxygen
fugacity of the upper mantle
Decades of study have documented variations of several orders of magnitude variation in the oxygen fugacity (ƒO2) of terrestrial magmas and of mantle peridotites. This variability is usually attributed to variations in the redox states of multivalent elements (e.g., Fe3+/Fe2+, S6+/S2-) in the mantle sources of magmas or to processes such as crystallization or degassing acting on magmas after segregation from their sources. Oli Shorttle (a former postdoctoral scholar, now a university lecturer at U. Cambridge), Paul Asimow (Caltech) and Paula Antoshechkina (Caltech) and I recently showed that the phase equilibria of plagioclase, spinel, and garnet lherzolites of constant bulk composition (including whole-rock Fe3+/Fe2+) can also lead to systematic variations in ƒO2 in the upper ~100 km of the mantle.
We used two thermodynamic models to calculate ƒO2 vs. P and T for a peridotite of constant composition (including total oxygen). As illustrated in Figure 1, under subsolidus conditions, increasing P at 1300°C in the plagioclase-lherzolite facies from 1 bar up to the disappearance of plagioclase leads to a decrease in normalized ƒO2 of ~1.25 orders of magnitude.
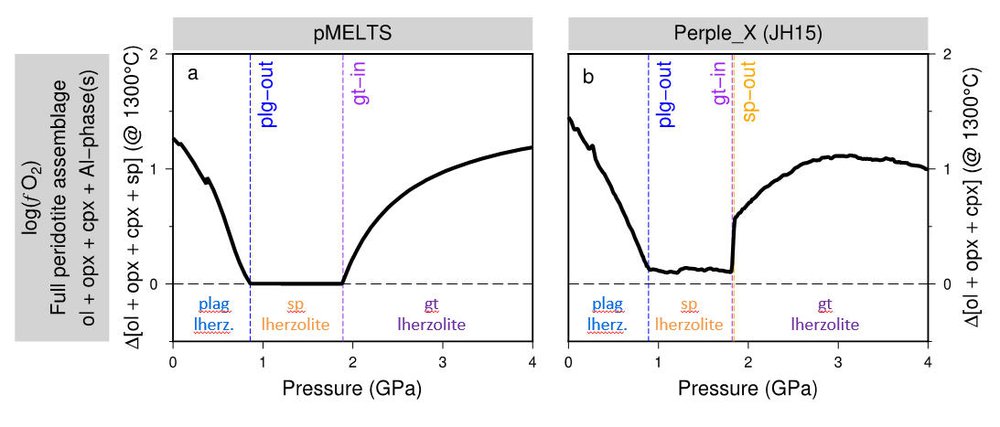
Figure 1. Calculated P-dependent subsolidus variations of log10ƒO2 for a representative mantle peridotite at 1300°C. These models predict large variations of ƒO2 in a peridotite of constant Fe3+/Fe2+ due to phase changes (i.e., from plagioclase lherzolite to spinel lherzolite to garnet lherzolite with increasing P). The panel on the left is based on the pMELTS model; the panel on the right is based on the "JH-15" model. The ƒO2 has been normalized so as to suppress variations that would occur in the absence of stabilization of plagioclase and garnet in the peridotite.
The spinel-lherzolite facies defines a minimum in ƒO2, and increasing P in this facies has little influence on ƒO2 up to the appearance of garnet. Increasing P across the garnet-lherzolite facies leads to increases in normalized ƒO2 of ~1 order of magnitude from the low values of the spinel-lherzolite facies. These changes in ƒO2 reflect primarily the indirect effects of reactions involving aluminous phases in the peridotite that either produce or consume pyroxene with increasing P: Reactions that produce pyroxene with increasing P (e.g., forsterite + anorthite Mg-Tschermak + diopside in plagioclase lherzolite) lead to dilution of Fe3+-bearing components in pyroxene and therefore to decreases in ƒO2, whereas pyroxene-consuming reactions (e.g., in the garnet stability field) lead initially to enrichment of Fe3+-bearing components in pyroxene and to increases in ƒO2. Thus, the variations in ƒO2 inferred from .modeling of upper mantle peridotite of constant composition are largely passive consequences of the same phase changes that produce the transitions from plagioclase → spinel → garnet lherzolite and the variations in Al content in pyroxenes within each of these facies. Since these variations are largely driven by phase changes among Al-rich phases, they are predicted to diminish with the decrease in bulk Al content that results from melt extraction from peridotite, consistent with our calculations.
Observed variations in normalized ƒO2 of mantle-derived basalts and peridotites within and across different tectonic environments probably largely reflect variations in the chemical compositions (e.g., Fe3+/Fe2+or bulk O2 content) of their sources (e.g., produced by subduction of oxidizing fluids, sediments, and altered oceanic crust or of reducing organic material; via equilibration with graphite- or diamond-saturated fluids; or due to effects of partial melting). However, our study showed that the predicted effects of P- and T-dependent phase equilibria on the ƒO2 of peridotite of constant composition are likely to be superimposed on variations due to bulk O2 content and therefore that the effects of phase equilibria must also be considered in efforts to understand observed variations in the ƒO2 of magmas and their mantle sources.
The behavior of volatiles in igneous processes
My colleagues and I have been studying for many years the behaviors (e.g., solubility, speciation, isotopic fractionation, thermodynamic properties) of volatile components (e.g., H2O, CO2, rare gases) as they relate to igneous processes on the earth and other planets. Recent examples include:
Degassing and its effects on ƒO2:
As mentioned above, degassing of magmas as they depressurize on ascent toward the surface lead to variations in ƒO2. The direction and magnitude of this effect depends on the concentrations of various volatiles dissolved in the melt phase, the ƒO2 of the magma prior to the onset of degassing, and the details of the degassing process. Former postdoctoral scholar Maryjo Brounce (now an assistant professor at the University of California, Riverside), John Eiler (Caltech), and I measured using XANES spectroscopy the Fe3+/Fe2+ and S6+/S2- ratios of a set of submarine basaltic glasses recovered as part of the Hawaii Scientific Drilling Project that experienced different degrees of degassing. Fe3+/Fe2+ and S6+/S2- ratios of the glasses decrease with decreasing concentrations of H2O and S (Figure 2), indicating that ƒO2 decreases continuously in Hawaiian magmas with progressive degassing by up to an order of magnitude; the observed trends can be matched using available thermodynamically based models of degassing.
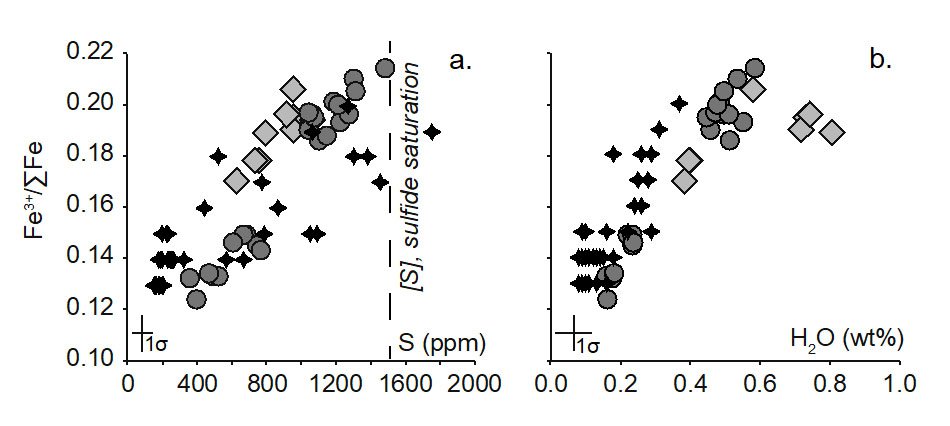
Figure 2. Variations of Fe3+/∑Fe ratio (measured by XANES) vs/ H2O and S abundances in Hawaiian submarine volcanic glasses. The Fe3+/∑Fe ratio decreases progressively with decreasing H2O and S concentration, demonstrating the decrease in ƒO2 (by ~1 order of magnitude) that accompanies degassing of Hawaiian magmas.
This reduction during degassing reflects the relatively high concentrations of sulfide-bearing species in Hawaiian melts and of S-bearing species in the melt and gas; degassing of H2O- and CO2-rich magmas is predicted to have smaller effects on ƒO2 (and most likely in the opposite direction) in agreement with observations on melt inclusions in from island arc magmas.
Diffusion and solubility of H2O (and H2) in silicate melts:
The solubility and diffusivity of water in silicate melts is critical for understanding degassing phenomena leading to the growth of bubbles and explosive eruptions. Megan Newcombe, a recent Caltech graduate (now an assistant professor at the University of Maryland) worked on water solubility and diffusivity of water in lunar basaltic liquids at the concentrations of water and oxygen fugacities relevant to eruption at or near the lunar surface. She was particularly interested in whether the highly reducing conditions of lunar basalt petrogenesis (near the iron-wüstite buffer) and the high partial pressures of H2 relevant to eruptions under these conditions affect water solubility and diffusivity and whether extrapolations of experimentally determined diffusion coefficients of water to the much lower water contents relevant to degassing into the near-vacuum at the lunar surface are valid.
As part of her Ph.D. thesis, Megan experimentally determined the solubility and diffusivity of water in a representative lunar basaltic liquid composition and in an iron-free analog of a basaltic liquid at the low water concentrations and low oxygen fugacities (fO2) relevant to the eruption of lunar basalts. Her work demonstrated that under the conditions relevant to lunar magmas: (1) hydroxyl is the only detectable dissolved H-bearing species; (2) as expected, the solubility of water is proportional to the square root of pH2O (Figure 3) and is independent of fO2 (i.e., pH2/pH2O); and (3) the concentration of H2 in the melts is <3 ppm, even at fO2's much lower than relevant for lunar magmas (pH2/pH2O ~ 11).
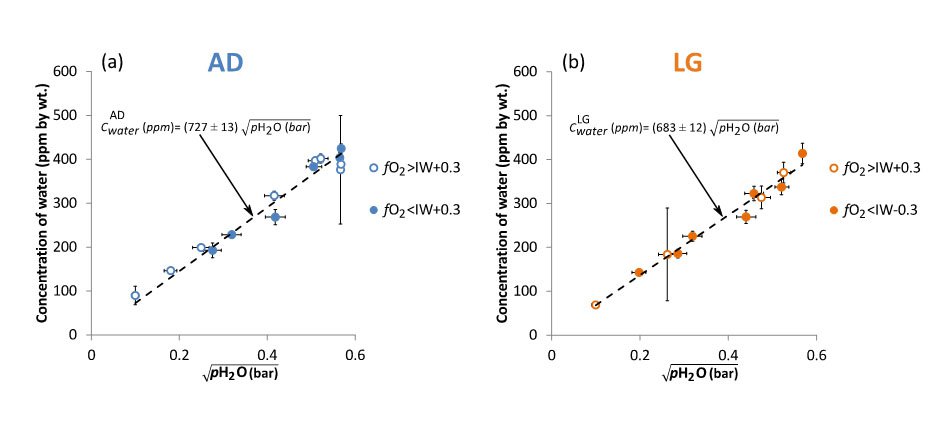
Figure 3. Demonstration of a proportional relationship between the concentration of water (measured by FTIR) in quenched melts and the square root of the partial pressure of water in gas with which they equilibrated mixtures at 1 atm). The AD composition (left panel) is a model basalt on the anorthite-diopside join and LG is a lunar basaltic glass composition. Note that there is no detectable effect of ƒO2 on the solubility of water.
These results can be used to constrain the entrapment pressure of lunar melt inclusions and the partial pressures of water and molecular hydrogen in the carrier gas of the lunar pyroclastic glass beads. The most water-rich lunar melt inclusion would be in equilibrium with a vapor with pH2O ~3 bar and pH2 ~8 bar, and the partial pressures of water and molecular hydrogen in the carrier gas of the lunar pyroclastic glass beads would be 0.0005 bar and 0.0011 bar respectively. These results lead to the conclusion that the vapor driving lunar fire fountaining is dominated by H2 rather than CO.
Water concentration gradients in the majority of the experiments designed to provide measurements of diffusion coefficients are well described by models in which the diffusivity of total water has a constant value (Figure 4).
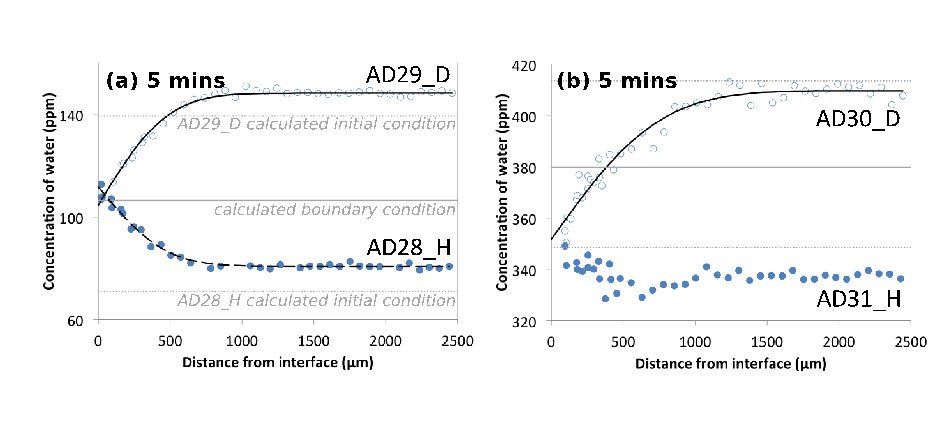
Figure 4. Example of results of water diffusion experiments on a model basaltic melt corresponding to the anorthite-diopside eutectic at 1 atm. Each panel shows two experiments run simultaneously: The upper sample was first equilibrated with a gas with a high pH2O and the lower sample was equilibrated at a higher pH2O, resulting a lower concentration of water; the two samples were then both equilibrated at an intermediate pH2O – resulting in the same boundary condition for both – and the sample with the initially higher water content lost water (producing a concave down water concentration profile) and the sample with the initially lower water content gained water (producing a concave up water concentration profile). Both samples can be fit with the same constant diffusion coefficient.
Our results are consistent with the conclusion that while diffusion of molecules of water dominate the flux of water at higher concentrations, the diffusivity of water is dominated by hydroxyl groups at the low water contents relevant to bubble growth and degassing of lunar basaltic liquids. Our results are also consistent with a positive correlation between the diffusivity of hydroxyl groups and melt depolymerization. Values of the bulk diffusivity of water in our experiments vary by less than a factor of ~2 over a range of pH2/pH2O from 0.01 to 10 and (a range of logfO2 from IW–2.2 to IW+4.9): The relative insensitivity of our best-fit values of the diffusivity of water over this large range in pH2 suggests that H2 diffusion does not control the rate of degassing of H-bearing species from the lunar pyroclastic glasses.
Chemical zonation in melt inclusions in olivine, plagioclase,
and pyroxene, and experiments and modeling to determine
cooling histories
It is generally assumed that glassy melt inclusions in phenocrysts are nearly homogeneous and thus that analyses from near the center of an inclusion are representative of the inclusion composition. This would seem to be a reasonable assumption, since even though crystallization of the host mineral on the walls of the inclusion and reequilibration of the melt inclusion with the host mineral are known to affect inclusion composition, the time scales for diffusive homogenization of melt inclusions are orders of magnitude smaller than residence times of phenocrysts in magma chambers.
As another part of her thesis, Megan Newcombe (working with me and John Eiler at Caltech, and several colleagues from other institutions, including Alberto Saal from Brown University and Youxue Zhang from U. Michigan) documented significant and ubiquitous zonation in naturally glassy olivine-hosted melt inclusions from the Siqueiros Fracture Zone and the Galapagos Islands; current graduate student Lee Saper found the same in samples from Hawaii and martian meteorites. Concentration profiles extend up to >100 µm into the inclusions, and have been documented for major, minor, trace, and volatile elements (Figure 5).
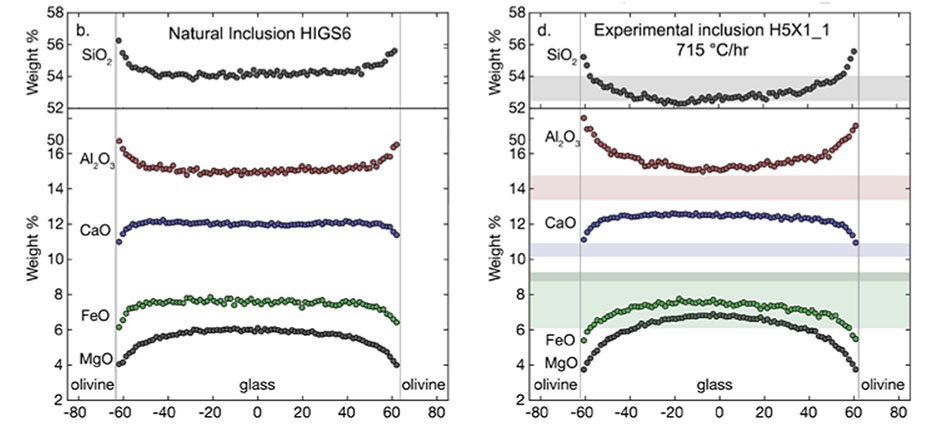
Figure 5: Microprobe traverses across a natural, unheated olivine-hosted inclusion (left panel) and an experimentally homogenized and then cooled inclusion (right panel) from Papakolea, Hawaii. The experimentally cooled and natural inclusions show similar features in the concentration profiles of all elements. The cooling rate experienced by both natural and experimental inclusions can be determined by fitting the observed MgO profile to a model of progressive crystallization of olivine on the wall of the inclusion and diffusion within the melt inclusion on cooling.
Components with a higher concentration in the host olivine than in the melt (MgO, FeO, Cr2O3, and MnO) are depleted at the edges of the zoned melt inclusions relative to their centers, whereas except for CaO, H2O, and F, components with a lower concentration in the host olivine than in the melt (Al2O3, SiO2, Na2O, K2O, TiO2, S, and Cl) are enriched near the melt inclusion edges. This zonation is due to formation of an olivine-depleted boundary layer in the adjacent melt in response to cooling and crystallization of olivine on the walls of the melt inclusions concurrent with diffusive propagation of the boundary layer toward the inclusion center.
Concentration profiles of some components in the melt inclusions exhibit multicomponent diffusion effects such as uphill diffusion (CaO, FeO) or slowing of the diffusion of typically rapidly diffusing components (Na2O, K2O) by coupling to slow diffusing components such as SiO2 and Al2O3. Concentrations of H2O and F decrease towards the edges of some melt inclusions, suggesting that these components may have been lost from the inclusions into the host olivine late in their cooling histories.
Megan also developed a simple model of the time-dependent evolution of MgO concentration profiles in melt inclusions due to simultaneous depletion of MgO at the inclusion walls due to olivine growth and diffusion of MgO in the melt inclusions in response to this depletion. Observed concentration profiles were fit to this model to constrain their thermal histories. Compositional trends with melt inclusion size are described well by this simple single-stage linear cooling model. Cooling rates determined by applying this model to the inclusions she studied are 150–13,000 °C/hr from the liquidus down to ~1000 °C, consistent with previously determined cooling rates for basaltic glasses. The zoning profiles and the best-fit cooling rates likely record late-stage cooling during syneruptive processes.
An important implication of this work is that compositions of zoned melt inclusions (even if measured at the centers of the inclusions) will typically have been diffusively fractionated relative to the initially trapped melt; for such inclusions, the initial composition cannot be simply reconstructed based on olivine-addition calculations, and caution should be exercised in application of such reconstructions to correct for post-entrapment crystallization of olivine on inclusion walls. Moreover, analyses of a melt inclusion at any point from the edge to the center of an inclusion can give results significantly affected by diffusion and from which the initial bulk inclusion composition cannot be simply determined.
Current graduate student Lee Saper has extended this work by conducting controlled cooling-rate experiments on olivine-hosted melt inclusions to simulate the development of compositional zoning observed in natural inclusions. He has been able to reproduce in these experiments (Figure 5) essentially all of the features of naturally glassy inclusions (including the complex effects of uphill diffusion), and most importantly, can retrieve the known cooling rates of the experiments (which ranged from 70–50,000 °C/hr), typically to within ±10% based on applying a modified version of the model used by Megan Newcombe (Figure 6).
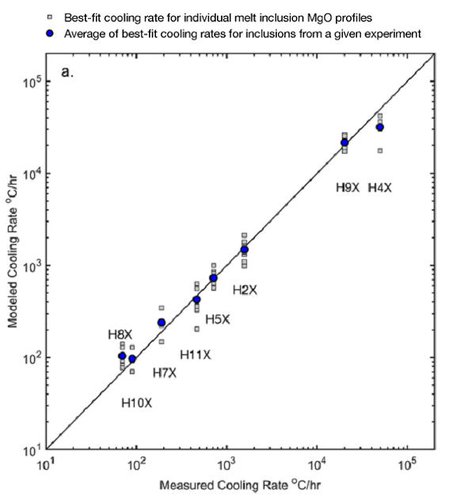
Figure 6. Comparison of cooling rates experienced by olivine-hosted melt inclusions during controlled cooling experiments and the best-fit cooling rates based on fitting their MgO concentration profiles. The model retrieves the known cooling rates to ~10% over several orders of magnitude.
These results demonstrate that fitting of the zoning patterns of natural inclusions based on this model can return accurate and precise values of cooling rates. He has also explored quantitatively the effects of cooling rate on uphill diffusion: CaO exhibits the most extreme manifestation of uphill diffusion, and a simple model attributes the diffusion behavior in CaO to solution nonideality in the boundary layer liquid.
Thermal histories of tektite impact glasses
Impact processes have had significant effects on rocky bodies in the solar system. On earth these include effects on the geological record, the environment, and life. Although theories of impact processes are sophisticated and useful, quantitative understanding of the histories of specific impact events is incomplete. A unique example of samples produced on earth via impact processes are tektites, which are natural terrestrial glasses, the origin of which (although at one time controversial) is now universally attributed to melting and quenching of distally ejected target material upon hypervelocity (>11 km/s) impacts. Former postdoctoral scholar Catherine Macris (now an assistant professor at Indiana University–Purdue University Indianapolis) worked with me and Caltech colleagues John Eiler and Paul Asimow plus James Badro (U. Paris) and Youxue Zhang (U. Michigan) to set quantitative constraints on the time-T histories of these objects that can be used to test and guide models of the impacts that produced them.
Tektites are composed primarily of felsic glass, but they contain inclusions of "lechatelierite", nearly pure SiO2 glass generally thought to form when quartz grains melted during the hypervelocity impacts that produced tektites. Given the high T (>1600-1700 °C) required to melt quartz, we hypothesized that diffusion likely occurred between the molten SiO2 and the felsic melt in which it was embedded. And indeed, when we examined these boundaries (Figure 7), we found that in all cases, the lechatelierite grains and the host felsic glass are separated by a chemically zoned, diffusively formed boundary layers (~100 µm wide) between lechatelierite and the host felsic glass.
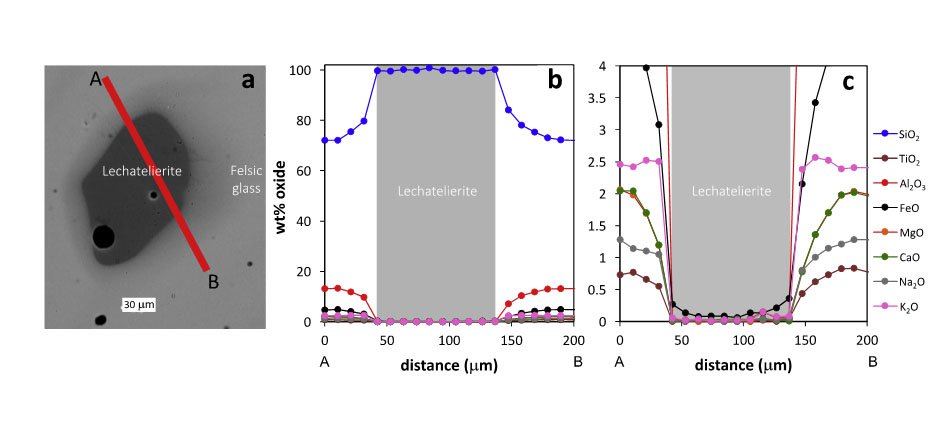
Figure 7. Typical concentration profiles of major elements across the boundary between lechatelierite grain and the surrounding felsic glass in a natural tektite: The left panel shows a back-scattered electron image of a lechatelierite inclusion (dark grey) surrounded by a diffusion halo that separates the lechatelierite from the far-field, lighter grey felsic glass. The middle and right panels show concentration profiles along the A-B traverse (note the difference in vertical scale between these two panels). Grey shaded regions in the right two panels indicate analyses inside of the lechatelierite grain; unshaded regions where the concentration profiles are changing represent the portions of the diffusion halo on either side of the lechatelierite. Where the concentrations level out, the far-field felsic matrix glass has been reached.
We were able to reproduce these boundary layers in detail (including uphill diffusion in K2O) via experiments on mixtures of powdered natural tektite and quartz grains that were exposed to temperatures of 1800-2400 ˚C for 1–120 s using an aerodynamic levitation laser-heated furnace in James Badro's Paris lab. The results of these experiments were then used to extract diffusion coefficients for various oxides in the observed boundary layers, which were in turn used to set quantitative constraints on the thermal histories of natural tektites.
If the interdiffusion between the SiO2 and felsic melts occurred at a constant T, the duration of heating experienced by a representative natural tektite from Thailand that we studied depends on the T; examples of possible solutions include heating at ~2000 °C for ~70 s, or -2400 °C for ~3 seconds. We also explored non-isothermal, asymptotic cooling histories: for a maximum T of 2400 °C, a characteristic cooling time scale of ~50 s is implied, whereas, for 2000 °C, the time scale is ~1400 s. Further, a maximum T of ~2360 °C would yield an effective diffusive time scale of ~5 s, leading to a cooling time scale of ~90 s, which gives a cooling rate at the glass transition T of ~5 °C/s; results that are consistent with independent estimates of cooling times for ~1 cm rhyolitic clasts in air and tektite cooling rates at the glass transition T – thus satisfying all currently available relevant data. More complex T-t paths are possible and they can also be modeled using our experimental results and compared with and used as tests of the accuracy of physical models of tektite-forming impact events.